When you give kids a glass of milk, they do not see clouds of cosmic dust in it, or colorful sunsets and blue sky. I don’t mean here the Carl Sagan’s “We're made of star stuff” quote, which points to the stars as the origin of atoms that make up the milk. I have in mind more mundane, easily visible effect of how light interacts with the fats and proteins in milk. In the today’s episode we will talk about a very simple, but important STEM experiment that every kid should try – light scattering in milk.
Milk is a fascinating liquid that played a big role in the rise of human civilizations. Or, if we want to be more precise, yogurt and cheese because these milk products reduce the amount of lactose, a type of sugar that adult humans couldn’t digest (but, lactose tolerance evolved extremely quickly and recently, most probably because milk was crucial for survival). The precise milk composition depends on various factors, but in general a cow’s milk is about 87% water, 5% lactose, 3.5% fat, 3.5% protein, and <1% minerals.
In our story we are interested in proteins and fat. Fat and water do not mix, which is why fat makes globule, small spheres, surrounded by other types of molecules (including proteins) that create a membrane separating the fat and water. In a raw fresh milk, the fat globules have the sizes between 1 and 10 µm. The milk proteins also combine together into tiny spherical aggregates, but their size is about 0.1 µm.
When I was a kid, we were buying fresh raw milk from our neighbors who had cows. Such a milk is a completely different experience than the milk we are buying in grocery stores. Not only that the taste is different, but if you leave a raw milk overnight, a thick layer of cream appears on the top. This enabled us to make our own cream and cheese. The reason for such a fast extraction of fat into a creamy layer is the large size of fat globules, which helps them flow more easily through water. This is why milk sold in stores first goes through the process of homogenization, where milk is pushed through a device that mechanically breaks the fat globules into smaller ones of sizes 0.2-1 µm. After that milk is pasteurized to eliminate pathogens and extend shelf life. Finally, it comes in stores with different amounts of fat, from whole milk (3.25% fat by weight), to reduced fat milk (2%), low-fat (1%), to fat-free (skim milk).
If you think that fat globules are so tiny that they are not visible by naked eye, you are right, but only partially. Actually, the milk is white because we see them! There are about 1 billion fat globules in one cubic centimeter of raw unhomogenized milk (and much more in homogenized milk). This number is so big that one drop of milk in a glass of water makes the water murky. The murkiness comes from the light being scattered toward your eye, which makes the completely transparent water suddenly visible. A few more drops of milk and the water becomes opaque because of too many fat globules preventing the light to pass through.
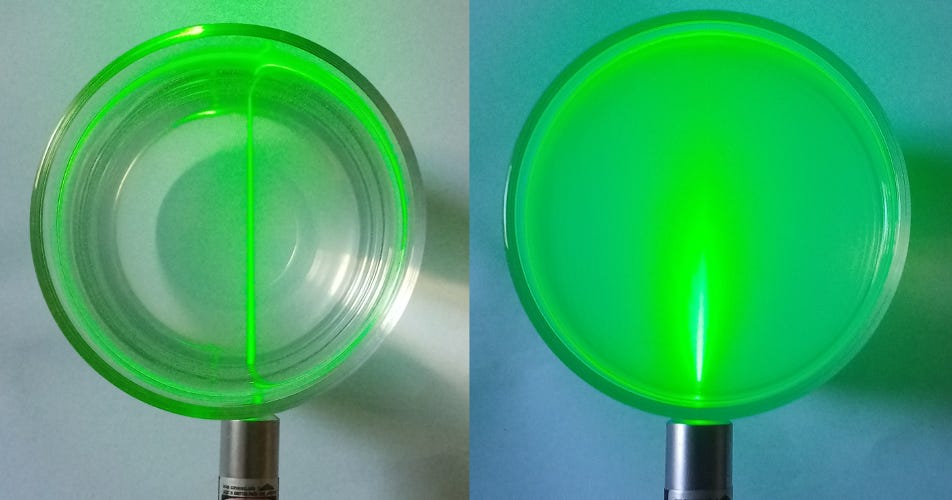
Interestingly, the same process of interaction between light and tiny spheres is happening in clouds. Unlike in milk where tiny drops of fat are immersed in water, clouds are spherical drops of water lingering in the air. The surface tension strongly keeps the drops spherical, where the size can vary a lot, but on average it is about 10-15 µm. The number of drops is now a few hundreds per cubic centimeter, which is much smaller than in milk. However, a cloud is much bigger than a glass of milk, and we are watching it from far away. This makes a cloud appear like a milk spilled over the sky.
The amazing thing is that we can extend this analysis to much, much bigger scales – to cosmic scales. The space between stars is not entirely empty. There is gas and dust that floats through spaces, on scales that take years for light to travel through. Sometimes these clouds collapse and form stars and planetary system, like ours. This is how that dust, initially created around dying stars, ended up creating Earth and all of us, which is the point that Carl Sagan made by “star stuff”. Space dust particles consist mostly of silicon, carbon, and oxygen atoms, but many other chemical elements are also present. The grain size is diverse, but below 1 µm, while their number is so small that in dense clouds, so dense that they block the light coming from the background stars (e.g., dark dense clouds), we expect only one particle within 1000 cubic meters (1 particle in a 10x10x10 meters box!). But these clouds are so immense that the total mass of all dust in the cloud is many times the mass of the Sun (and much more mass is in gas). And still, despite such a grandiose scale, we can observe light scattering like in a glass of milk (e.g., tenuous clouds around Pleiades or dark cloud Barnard 86).
There is, obviously, something generic about the properties of light scattering if we can use a similar analysis in all these cases. Indeed, the first impression is that scattering is like a particle bouncing off of a target, but this is not how it happens. We need to think of light as a wave if we want to dig deeper into the physics of scattering. In our previous episode we talked about the wave nature of light and how it is necessary for explaining rainbows. Light is a wave of oscillating electric and magnetic field, and when these traveling oscillations encounter a small obstacle (target), a complicated interaction follows. Atoms in the target participate in creating electric and magnetic fields within and outside the target. The whole interaction is a messy dance of electricity and magnetism that we observe from a very large distance. What ends up in our eyes is the end result of this dance, with different intensities of light being emitted into different directions around the target (similar to light interference described in our previous episode). Details depend on the target’s physical and chemical properties (shape, composition), and the frequency (i.e., wavelength) of light wave oscillations.
Advanced solutions to this problem emerged in the end of 19th century, while a complete mathematical tool for solving scattering on homogeneous spheres was published by the German physicist Gustav Mie in 1908. Even though this was just a special case of scattering, it opened a myriad of applications and paths for further improvements. Today, there are whole branches of science dedicated to the details of this problem, from both the experimental and theoretical perspectives. Just to get a feeling on how light scattering is complicated and beautiful, consider that by the end of 2021, scientists registered 119 types of halos in the Earth’s atmosphere formed by light (from Sun, Moon, or artificial sources) scattered on mostly ice crystals.
However, as we noticed, there are still some generic properties, something that can be applied to scattering in broad terms. This is where we introduce our simple experiment:
Prepare a glass bowl, milk, and flashlight. Fill the bowl with water and put it in front of a white surface.
Shine the flashlight through the water onto the white surface. Notice the whiteness of the light.
Add a drop or two of milk into the water (stir it to dissolve it). Turn on the flashlight again and check if you see any change in the light color.
Add milk drop by drop and monitor the light color on the white surface. Compare this color with the color of the murky water. Keep doing this until no light passes through.
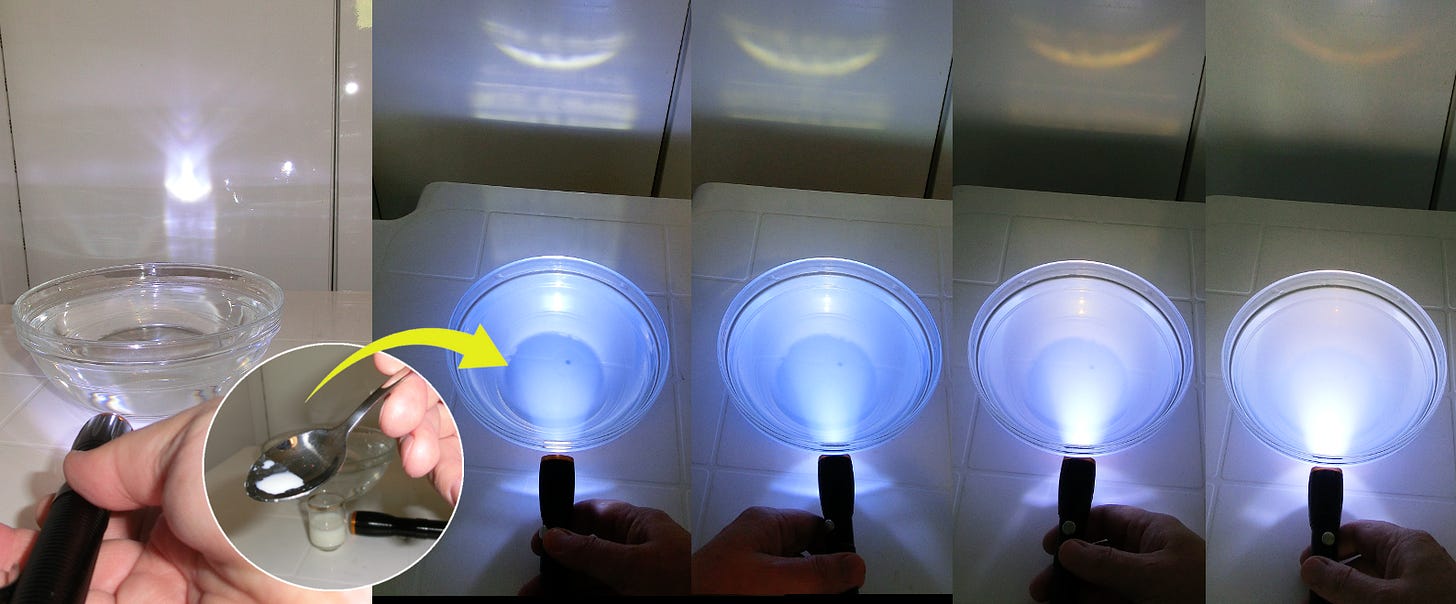
In a previous episode we described experiments that show how while light consists of a rainbow of colors. Now you will notice how the red component of white light has the ability of penetrating the milky water much easier than the blue component. After a few drops of milk, the water starts to glow blue, while the light that exits the bowl is reddish because it lost its blue component. The ability of blue to be scattered more easily than red is hidden in the general properties of scattering. When the size of targets is comparable or smaller than the light wave wavelength, the shorter wavelengths are scattered more strongly than longer. Blue has wavelengths of about 0.45 – 0.5 µm, while red has about 0.63 – 0.74 µm. Homogenized milk has fat globules about that size or smaller, and protein aggregates even smaller. These are just the right conditions to notice this scattering effect. Once you add too much milk, the liquid becomes white because all wavelengths (i.e., colors) are scattered now, and they mix back into white. This is why milk is white, and clouds in the sky, too.
The effect of blue color getting removed from the rainbow of light passing through the milky water is nicely visible in the following experiment:
For this you need a larger glass tank (like a fish tank) and a flashlight. Use aluminum foil to make a cover with a narrow vertical slit for the flashlight.
Fill the tank with water and add some milk to make it slightly murky. Put the flashlight with slit on the one side of the tank and observe it from the other side (B in the image below). You will see it has a reddish color.
Now position the light source in such a way that the light beam enters the tank in a shallow angle and exits through the sidewall (paths C in the image below). This is a setup that we already used for creating a rainbow thanks to different colors taking a slightly different path. The fun part here is that if you move your hand a little bit left-right, you will notice how colors in the rainbow change. Paths with longer distances through the murky water have a noticeable reduction in the intensity of blue color.
These experiments sound trivial, but when the whole thing is done using high precision instruments and professional equipment, the scattering effects can be used for measuring the fat and protein concentrations in milk.
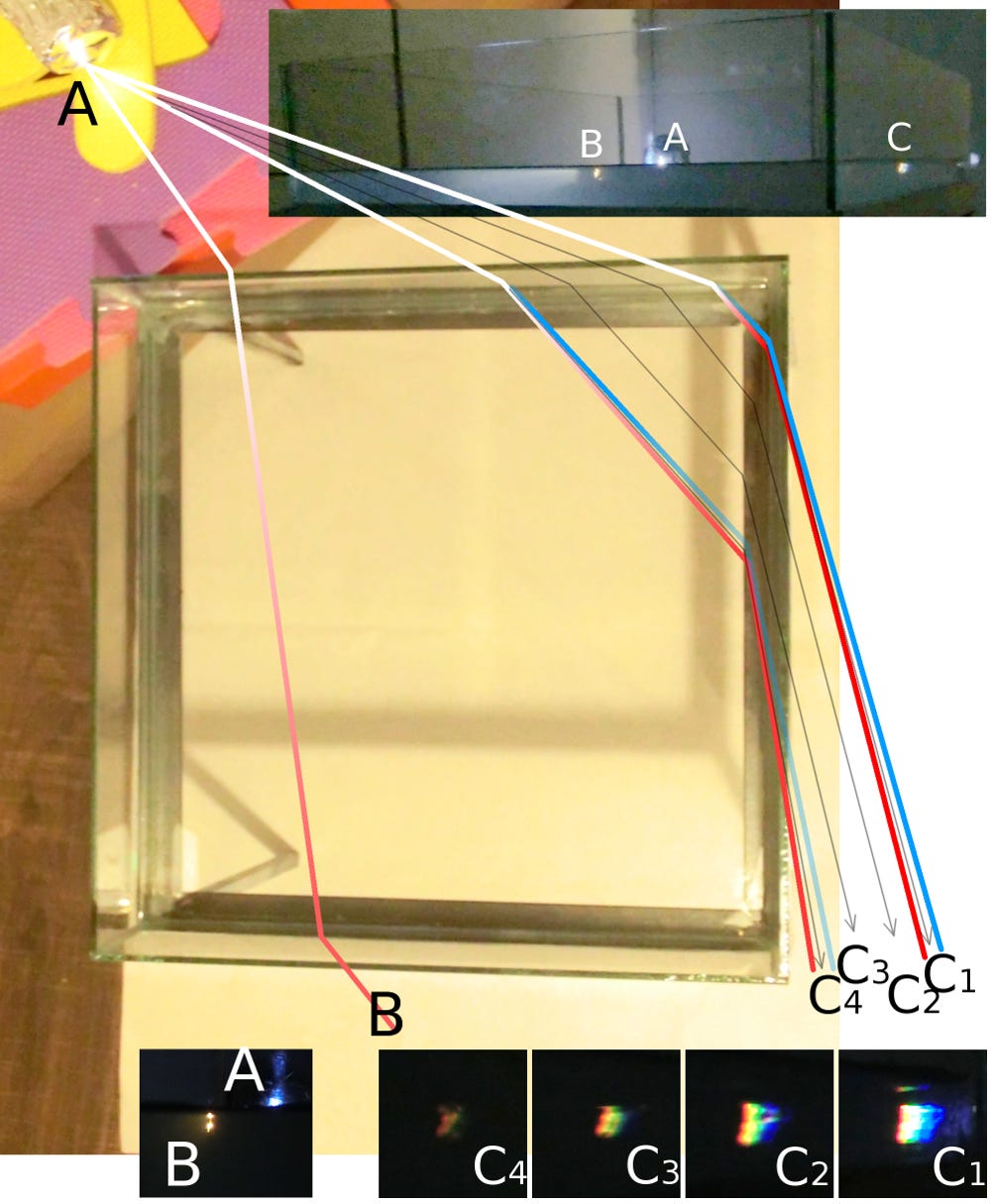
You might wonder what happens if we use even longer wavelengths of light than red. Such colors, called infrared, are not visible by humans, but specialized cameras can detect them. As expected, scattering is reduced, and these invisible colors can pass through more easily. This trick is used by firefighters who use cameras for infrared vision to see through smoke. Smoke are particles of about 1 µm, and infrared light is not scattered significantly. A series of images below demonstrate this. Exactly the same approach is used by astronomers who use images in different infrared colors to see how a dark space cloud gets transparent, and from this they deduce the properties of space dust.
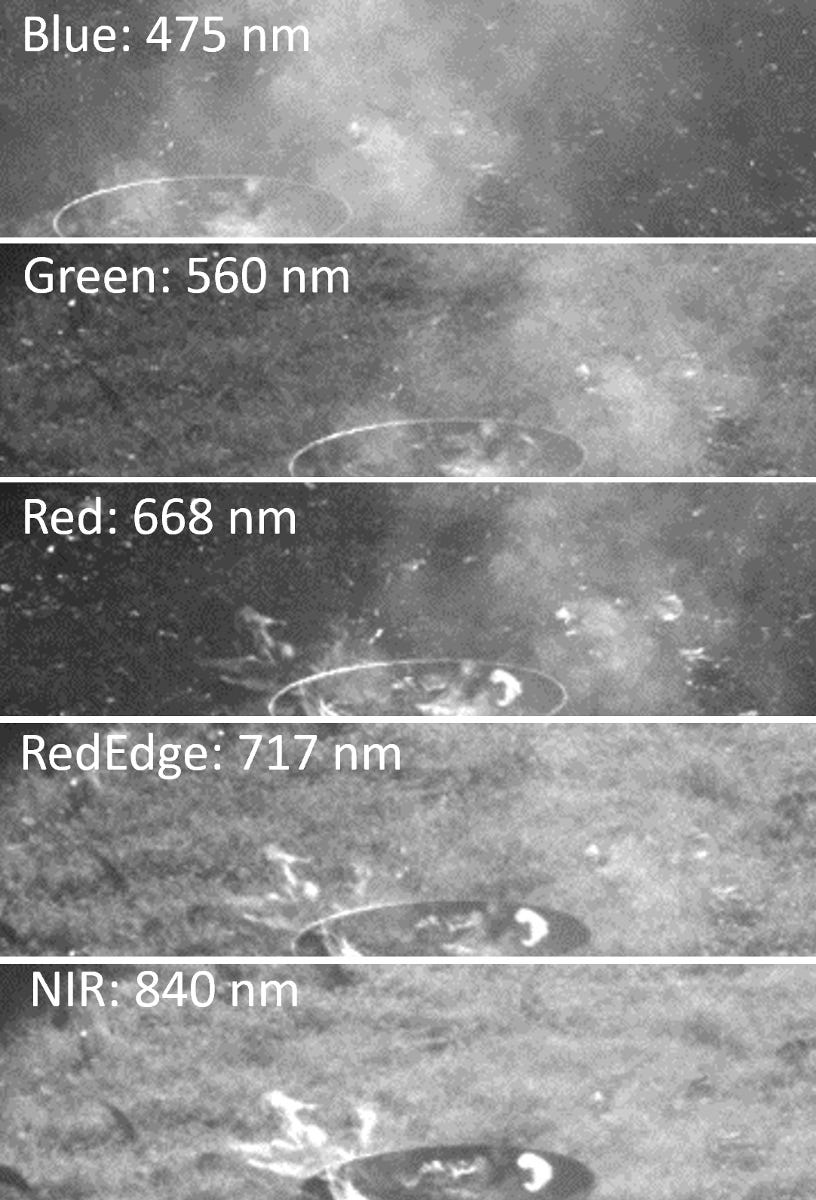
The examples so far are in the domain where the particles are not much smaller than the light wavelengths. We notice that scattering is somewhat stronger in the blue range, but it is not a strong effect. This is the domain of so-called Mie scattering. It becomes more dramatic when the particles are much smaller, like in the case of air molecules in the Earth’s atmosphere. The sky is like a big bowl of milk, but with a more extreme case of blue scattering compared to red. This extreme regime of scattering is known as the Rayleigh scattering (named after the 19th-century British physicist Lord Rayleigh).
Interestingly though, it is not that the sky has no other colors. Violet color has a shorter wavelength than blue, and it is also strongly scattered. Other colors also exist in the mix, albeit with a lower intensity. But we see blue even though this is not like watching a blue ink where other colors do not exist. It turns out that our visual system (eyes and brain) cannot distinguish between a pure blue and a mix of violet, blue and white. We talked in our newsletter about this type of tricks that our brain plays with us and how magenta color does not exist.
Similarly, when we watch light passing through lots of atmosphere, like during sunsets, we see how it becomes orange and red. Humidity, small droplets, dust, and aerosols (tiny particles) contribute to the reddening effect in the same way as we explored in the milk experiment. The size of particles influences the intensity of red colors. This effect can be nicely seen in the Bishop's Ring halo caused by the Sahara desert dust in the atmosphere. Volcanoes are also a source of particles that travel large distances and produce intense red sunsets, like recently by the Hunga Tonga-Hunga Ha’apai volcanic eruption.